The conversation in quantum computing has shifted from “if” these machines will do something useful for society to “when”.
But to unlock the true potential of quantum computers, they need to get bigger. Today’s quantum computers are of the order of dozens of quantum bits (qubits). To start to do anything useful with quantum computers, we need to reach the million-qubit scale.
It’s a monumental task but not an impossible one with the right engineering focus to scale to the million-qubit range.
What are qubits?
While traditional computer bits store information as a 1 or a 0, qubits can exist in multiple states at the same time, in a so-called superposition between 1 and 0.
This quality gives quantum computers their immense power, allowing them to complete complex calculations that are not possible on traditional machines.
But qubits are also incredibly fragile. Even the slightest amount of noise can cause the underlying quantum information to break down, rendering the quantum computer useless. Controlling these qubits is the crux of the scalability issue and quantum computing hardware companies are faced with four key challenges to address.
Engineering Challenge #1: Choosing qubits
There are many ways to make a quantum computer and one of the first decisions is the choice of qubit to use. There are many types of qubits, each with its own set of pros and cons. Superconducting and trapped-ion systems are two of the main contenders.
Superconducting machines essentially use a tiny superconducting circuit to form each qubit. Their basic components are compatible with classical chip technology and IBM’s 127-qubit quantum computer (the largest in the world at time of writing) uses superconducting technology.
But as we scale further, trapped ion systems are starting to break through thanks to their less stringent refrigeration requirements, improved connectivity and long coherence times.
Trapped-ion systems use individually charged atoms – ions – as their qubits. These trapped ions are naturally identical, well isolated from the environment and easily controllable. They also provide superior connectivity where (with the right architecture) you can move qubits to ‘talk’ to one another.
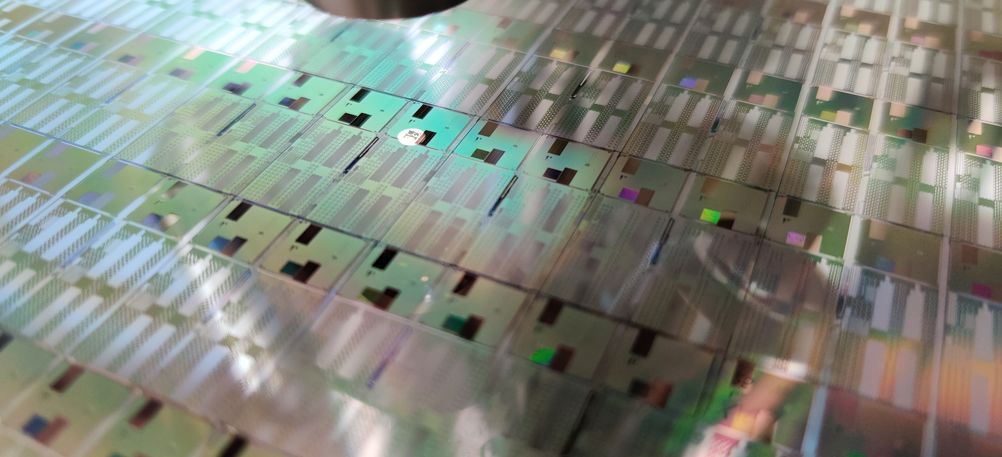
This high connectivity may allow more efficient quantum error correction methods to be used. Practically this means fewer qubits can provide the same computational power. Superconducting qubits on the other hand are immobile entities and only have nearest neighbour connectivity.
But it’s not a clear-cut case. Superconducting systems have faster gate times, allowing them to complete calculations faster than their trapped ion counterparts. But trapped ion systems have longer coherence times (the duration you can maintain a stable position of a quantum computer).
There are also many other considerations as we start to scale these machines.
Engineering Challenge #2: A temperature that scales
To maintain the fragile quantum states of qubits, quantum computers need to operate at low temperatures. This exact temperature is dependent on the type of qubit used.
Superconducting machines require cooling to near absolute zero. As we scale, the refrigeration requirements become increasingly challenging as these machines grow in scale and complexity.
But trapped ion systems do not require such stringent refrigeration requirements, which is important as we scale up the number of qubits. Our trapped-ion technology only requires cooling to 70K (around liquid nitrogen temperatures), for example.
Engineering Challenge #3: Lose the lasers
To complete a quantum calculation, you need to run a quantum circuit. To achieve this, quantum gates manipulate qubits in the quantum computer. Again, there are many ways to achieve this.
Trapped ion quantum computers generally use pairs of laser beams that must be aligned to the position of individual ions to execute quantum gates. This requires alignment accuracy to a fraction of the width of a human hair. But imagine trying to align millions of laser beams with such incredible accuracy when we reach the million-qubit scale, while stabilising each laser’s power, position and phase – in the knowledge that the slightest deviation would stop the quantum computer from working.

As we scale, simpler electronic gate technology is required. For example, our quantum gates use global microwaves, effectively creating radio stations. Want to do a single qubit gate? Apply voltages to tune into that channel. Want to do a two-qubit gate? Apply a different set of voltages to tune into that channel. The best part of this gate scheme is that it doesn’t matter if we have 10 or 10 million qubits, the number of microwave sources is fixed allowing for dramatically simplified scaling.
Engineering Challenge #4: Fully integrated modules
With a single wafer holding just a few thousand qubits at most, modularity is critical to scale quantum computers to the million-qubit range.
One proposed method for achieving this uses photonic interconnects, with optical fibres connecting different quantum computing modules. After several years of research, the connection speeds for this technology remain slow and add significant practical overheads to implement. This could therefore provide an insurmountable bottleneck when scaling up.
We take a completely different approach to modularity, which uses electric field links between adjacent quantum computing modules. As well as using much simpler engineering, it will enable connections between modules that are orders of magnitude faster.
Also, by developing fully electronic quantum computing modules based on integrated silicon microchips, we can capitalize on the wealth of expertise that has come out of the development of the silicon microchips used in conventional computers. The result is fully integrated self-contained electronic quantum computing modules – a must for any quantum computer to scale.
Are we nearly there yet?
In truth, the hard (and exciting) work in quantum computing is just beginning. As these machines start to scale and move out of the world’s laboratories, these engineering problems need to be resolved. While it’s a mammoth task, it’s not an impossible one – with a practical engineering approach.
And that’s when the truly exciting conversations about quantum computing can start as we don’t just start to understand “when” but also “how” quantum computing will impact society.
Zak Romaszko is quantum chip lead at Universal Quantum
Invinity to build 20MWh flow battery in UK
Redux flow cells have had nowhere near the R+D effort compared to Lithium ion. They certainly have the potential (pun not intended) for longer...